Gas Fermentation of Cupriavidus necator H16 at Elevated Pressure
Dr. Tuck Seng Wong a, Dr. KangLan Tee a, Dr. Jasbir Singh b, Dr. Mark Appleton b
a – University of Sheffield, Sheffield, S1 3JD
b – H.E.L Ltd, 9-10 Capital Business Park, Borehamwood, WD6 1GW
Highlighting the use of an H.E.L BioXplorer system to conduct fermentation of Cupriavidus necator H16 at pressures between 1 to 4 bar, using either CO2 or sugar as the sole carbon source.
Table of Contents
Abstract
In this case study, the H.E.L BioXplorer was used to evaluate microbial fermentation in either autotrophic mode or heterotrophic mode. In autotrophic cultivation, elevated gas pressure allowed for process economics to be directly improved through higher biomass production rates. Substantial increases in gas solubility and gas-liquid mass transfer were demonstrated while at the same time achieving a fine control of dissolved oxygen profiles. This has applications targeting both bio-fuels and bio-alcohol production. Operating at a range of fermenter volumes from typically 100 ml upwards and working pressures of up to 60 psi (~4 bar), the biomass production rates for autotrophic cultivation using CO2 /H2 as gas feed were comparable to that for heterotrophic cultivation with gluconate as the carbon source.
Introduction
The commercial feasibility of many bio-processes can depend on how fast the gas transfer takes place. This is especially true if gas solubility is poor. For example, when working with gases such as hydrogen and methane in the context of gas fermentation for the production of fuels and chemicals from waste gases, the engineering solution for poor gas transfer is often limited to increasing kLa through changes in sparging and stirring arrangements. This offers a very limited scope for improvement; therefore, many potentially interesting processes can be rendered uneconomical. A much more effective alternative is to operate the bio-reactor at elevated pressure as this can, in principle, increase gas transfer rate several-fold without any changes to sparging or agitation.
There are still challenges to overcome to further increase the growth rates to justify the investment in scale-up use. These include, but are not limited to, the low density of gases, low gas solubility (CH4, H2, O2 ~1 mmol/kg water, and CO2 ~ 30 mmol/kg water) and slow C1 gas transfer rates, which will all limit the net conversion rate. Increasing the pressure of fermentation will help speed up the gas transfer.
Equipment and Methodology
Cupriavidus necator is a widely recognized converter of C1 sources into a range of different organics. Substrate conversion is dependant on the specific strain and the genetic modification of the strain. The strain used in this study is C. necator H16, a non-pathogenic bacterium commonly found in soil and freshwater habits with intrinsic ability to produce polyhydroxyalkanoate bioplastics. This strain grows with both gluconate and CO2 as carbon sources. Because of this, the fermentations were performed under both heterotrophic and autotrophic conditions.
A total of 10 fermentation conditions were tested as summarized in Table 1. Unless otherwise stated, fermentations were performed at 30 °C using an inoculum prepared in synthetic medium MSM with 1 % sodium gluconate. The fermentation broth was inoculated to OD600 = 0.3 at the start of cultivation. The fermentation broth was controlled at pH 6.8 using 0.2 M NaOH and 0.2 M H2SO4.
The BioXplorer was set up to monitor pH (probe: Hamilton, Polilyte Plus XP), dissolved oxygen (probe: Hamilton, VisiFerm DO Arc 120), turbidity (probe: Anglia Instruments, Turbidity Probe SS316), temperature (probe: Helium, PT100 Class A 4-wire), and biomass (probe: ABER, FUTURApico[C1] ).
All parameters were continuously monitored and automatically adjusted via H.E.L’s WinISO software. All data shown apart from the calculated OD600 value has been exported from the WinISO software.
Results and Discussion
Cell growth was observed in all the heterotrophic fermentations (run #1-#5), apart from #3 which was run under 4 bar conditions, with no viable cells in the final culture. The maximum growth rate observed was 0.326 h-1 from fermentation #4 (Figure 1). HPLC analysis showed the gluconate was completely consumed at the end of the fermentation.
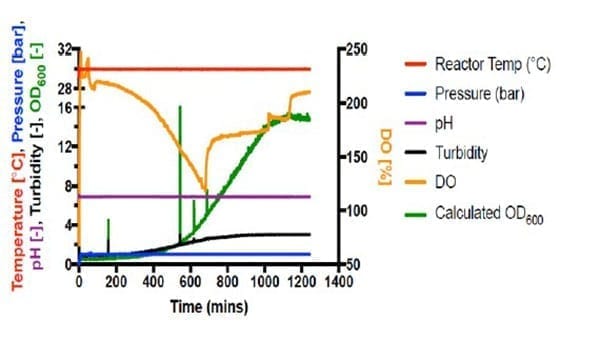
Autotrophic cultivations at pressure were achieved by increasing the pressure in steps of 1 bar and held for 20 minutes following each step increase. Fermentations #6 (Figure 2) and #7 (Figure 3) are shown below.
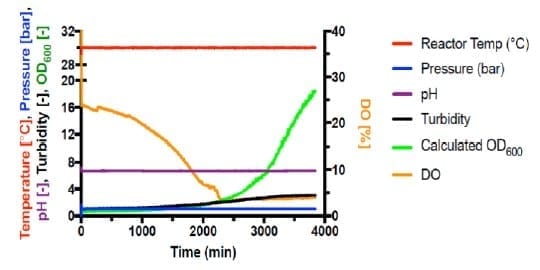
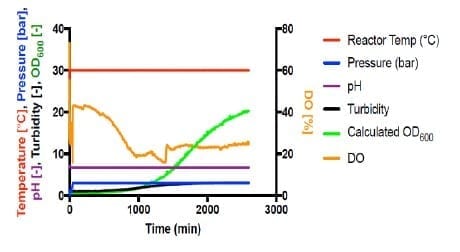
Shown in Figure 4, all autotrophic growth rates were slower than that of heterotrophic cultivation at 1 bar (#4). Increasing the pressure from 1 bar to 3 bar increased the growth rate by 166%. However, increasing pressure to 4 bar significantly decreased the growth to 24% compared with 1 bar. This suggests that the barotolerance of C. necator H16 is likely around 4 bar. Further work would be necessary to increase the barotolerance of C. necator using laboratory evolution, which can also be conducted using the BioXplorer.
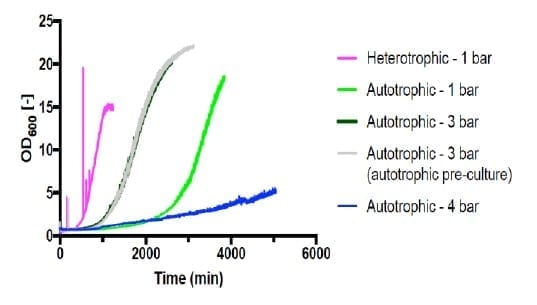
Conclusion
The H.E.L BioXplorer was used to successfully investigate the effects of pressure on the growth of C. necator H16 under both heterotrophic and autotrophic conditions. Growth under heterotrophic conditions remained feasible even when pressure was increased from 1 bar to 3 bar. [C1] This is not surprising, as the carbon source of gluconate was readily available at all pressures. However, under autotrophic conditions, pressure up to 3 bar significantly increased the growth rates up to 166% when compared with 1 bar.
The benefits of running fermentations under pressure is clearly shown with the H.E.L BioXplorer. It is therefore possible that using elevated pressure for microbial fermentations makes autotrophic growths economically viable.
Acknowledgments
We would like to thank Dr. Wong, Dr. Tee and their teams for completing this, and for sharing this data with us. Our research collaboration with The University of Sheffield is supported by BBSRC C1Net BIV, BBSRC IAA, and the RAEng|The Leverhulme Trust Senior Research Fellowship (awarded to Dr. Wong with H.E.L. as an industrial partner).