The Thermodynamic Foundation
“In this house, we obey the laws of thermodynamics” Homer proclaimed in one of the episodes of The Simpsons. In all its complexity and diversity, from bacterial cells to human society, life adheres to the fundamental laws of thermodynamics. Understanding how organisms harness and manipulate energy to sustain and replicate themselves is fundamental in order to unveil the very essence of life.
At its very core, biothermodynamics rests upon the principles of classical thermodynamics. The first law states that energy cannot be created or destroyed, only transformed. Enthalpy is derived from this principle and can be defined as the heat subtracted or added by a chemical process at a constant pressure. The second law determines that for a process to occur spontaneously, it needs to increase the entropy of the universe. Rounding up, the third law of thermodynamics states that the entropy of a system approaches a constant value as its temperature approaches absolute zero.
Generally, exothermic reactions (processes that release energy and therefore have negative enthalpy values) happen spontaneously. However, there are cases in which endothermic reactions occur without external energy supply. In these cases, the entropy is the driving force. Gibb’s Free Energy is the parameter that ties these two thermodynamic values through the equation:

Where ∆G is Gibb’s Free Energy, ∆H is the enthalpy of the process, T is the temperature, and ∆S is the variation of the entropy of the systems. Negative ∆G values denote exergonic process that happen spontaneously, whereas positive values require the supply of external energy for the process to occur.
Cells as Thermodynamic micro-machines
Living organisms have the ability to exploit chemical gradients to produce energy. Certain organisms can utilize light in order to harness the energy required for their metabolism (photosynthesis). The other approach uses reduction-oxidation (redox) reactions, deriving energy from electrons transferring between the members. The most immediate and common way to store this energy is to form a chemical bond between adenosine bi-phosphate and a phosphate ion, generating adenosine triphosphate (ATP). This bond is reversible, and the ATP molecule can be cleaved when energy is required.
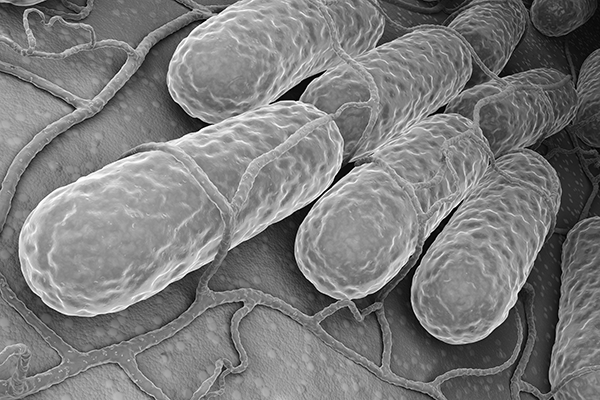
Metabolic reactions can be split into two categories:
- Catabolism: it encompasses chemical reactions in which complex molecules are broken down into simpler components, releasing the energy trapped in the chemical bonds in the process.
- Anabolism: it includes metabolic pathways that result in the synthesis of molecules from their smaller building blocks. In this case, energy needs to be supplied.
Understandably, the Gibb’s free energy and the substrates available to organisms will determine which metabolisms are viable. Different chemical reactions will yield different energy outcomes that will power the cells. For example, the oxidation of glucose in the presence of oxygen releases a vast amount of energy, which will result in aerobic heterotrophic organisms having very low doubling times (the time that it takes a microbial culture to double the number of members). Other reactions are not as efficient, as we can see in the redox tower,
E is the electric potential of the reaction, which is defined as the energy per unit charge available from the oxidations/reduction reaction. The relationship between the electric potential (E) and the Gibb’s Free Energy is very straightforward and it is captured by the equation:
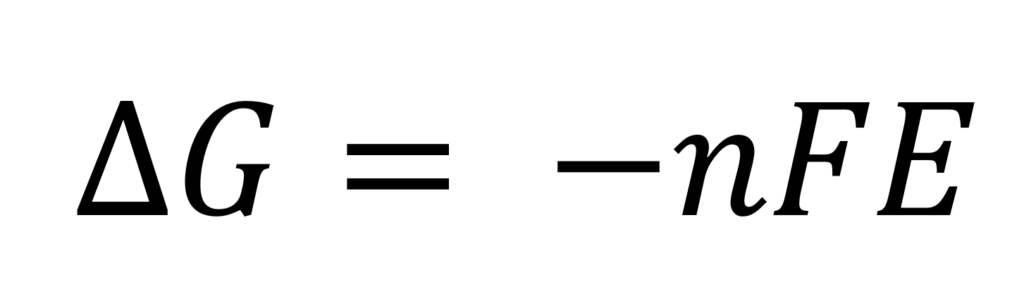
Where n is the number of electrons transferred and E is F is the Faraday constant 96,485J/(V⋅mol).
Gibb’s Free Energy and Cellular Growth
Chemical reactions have been extensively characterized thermodynamically in order to build tables that contain values such as enthalpy, entropy, and Gibb’s free energy. However, cells, due to their intrinsic complexity, have fallen behind. Nevertheless, efforts have been made to try to characterize the properties of microbial biomass.
In this pivotal work, we can see different ∆G values for different microbial species. The immediate conclusion for this work is that, for cells to replicate themselves, they would need to allocate energy to synthesize new biomass. The energy required for such a process should then derive from the redox reactions we mentioned before. The yield of these reactions will then determine how much energy is available and if it is viable for the cell to replicate. Recent studies have aimed to try to find the relation between Gibb’s free energy and the microbial growth of microorganisms, highlighting the importance of thermodynamics in life.
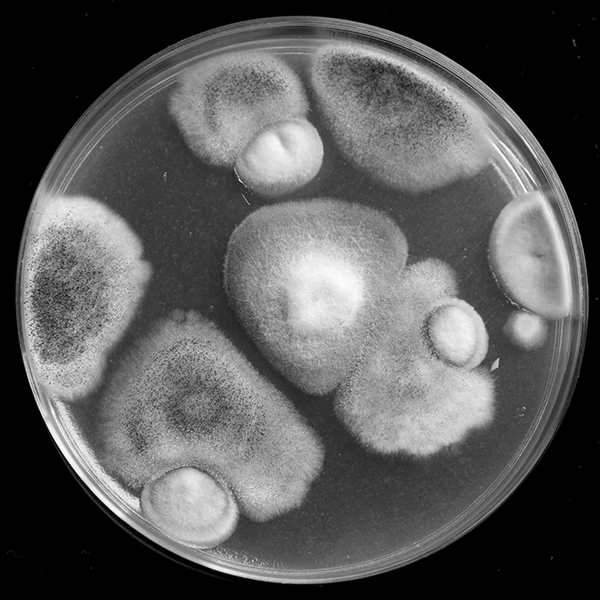
Why Unravelling Biothermodynamics Matters
Despite being a very complex tapestry of interconnected chemical reactions contained in cells, life is governed by the same fundamental principles as fundamental chemistry. In its fight for survival, laws such as that energy is neither created nor destroyed and that the universe tends towards entropy lay the ground rules for life. Gibb’s free energy emerges as a critical player, determining the spontaneity of these chemical reactions. Organisms, as tiny biological machines, have the ability to harness the energy released by spontaneous chemical reactions to drive their metabolism.
This is why it is fundamental that we unravel biothermodynamics. The ramifications of this knowledge are vast. It will help us understand how much organisms can grow. It will provide us with tools to optimize our processes, redirecting the energy allocation of cells into products that we could be interested in. But it can also provide information about in which kind of setting organisms can grow, opening the gates for fields as exotic as astrobiology. Tools such as adiabatic calorimetry and simulation can be of unmeasurable help for this.