At H.E.L, there’s nothing we don’t know about the capabilities of the scientific instruments and software we develop and manufacture. Plus, we can share loads about how they optimize bench-level efficiency, safety, and productivity. But like you, we know it’s the difference our innovations are making to the quality of real-world science right now that is one of their most exciting features.
That’s why we are excited by chemists from Virginia Tech, led by Dr Amanda Morris. They’re applying our high-pressure catalysis system, the DigiCAT, to innovative artificial photosynthesis techniques to transform captured CO2 into viable plastic and fuel alternatives. We’re grateful to them for this fantastic insight into what catalysis in action in their hands is achieving.
- Catalysts can surprise you
- Measuring those mixed materials
- Artificial vs. natural photosynthesis
- Sourcing the right solution
- Catalytic futures for a greener planet
Catalysts can surprise you
The molecular stability of CO2 is no help when redirecting excesses of the greenhouse gas to produce alternative resources. A catalyst capable of making it easier and more economically viable to convert the gas into other useful chemicals is needed to combat this. Far easier said than done, it seems. The reality is that it takes masses of energy to break down CO2.
The most efficient catalyst for CO2 reduction, somewhat surprisingly, is metallic copper. A typical feature of a catalyst is that it must stand up to being used repeatedly, with a ‘turnover number’ as high as possible. The turnover number is defined as the number of molecules of substrate that can be converted by the active site of an enzyme when a substrate saturates the enzyme. Industrial catalysts require a turnover number in the millions to be economically viable. Dr Morris’ group is working on improved catalysts to replace metallic copper with a molecular structure, but the current turnover numbers are only in the 10s. Although this aspect is not perfect, other benefits of the catalyst mean the team finds it effective enough to persist with.
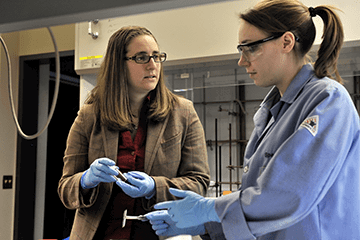
Measuring those mixed materials
Returning to metallic copper, while a very energy-efficient catalyst for CO2 reduction, copper is non-selective and will generate as many as 32 products in a mixture. This then creates an extreme engineering puzzle to separate and purify those products. Dr Morris’ molecular approach enables her group to select a single product despite current low turnover numbers.
One of the challenges across the field of catalysis is the difficulty of characterizing surface chemistry during the catalytic reaction. The reaction happens at the catalyst’s surface and may involve several intermediates before the product is formed. Few scientific techniques are available to probe molecular chemistry at the surface, and the surface is a complex environment. The surface of the copper metal is heterogenous: the atoms forming the surface will be bonded to the bulk of the copper in different ways, meaning they have differing availability for catalysis and potentially offer differing catalytic pathways. At an atomic level, researchers need to fully understand how many heterogenous catalysts work, including copper, for CO2 reduction. This means new catalyst formulations must be trialed and cannot simply be designed on paper.
Heterogenous catalysts consist of catalyst nanoparticles, often metallic, on a support, usually an oxide. Since all catalysis occurs at surfaces, nanoparticles, due to their small size, present an ideal surface-to-volume ratio and can be used to maximize the surface area of the catalyst component. The support keeps the nanoparticles in place, contributing to high turnovers. The metal-organic frameworks (M.O.F.s) used by Dr Morris’ group are the molecular equivalent of the nanoparticle-support structure. The copper nanoparticles are replaced by nodes of copper atoms connected by organic links (ligands), which act as the support. These catalytic ligands form extended 3D solid-state polymer structures or synthetic handles that improve catalytic effectiveness and energetics. Plus, and very importantly, the M.O.F.s can be separated from a solution in a centrifuge. The presence of these M.O.F.s increases the impact of copper as a molecular catalyst, offering a combination of stability and selectivity.
Artificial vs. natural photosynthesis
The M.O.F.s are also proving hugely useful in the team’s efforts to interpret nature’s relationship with CO2 during artificial photosynthesis (read about this work in our dedicated blog post) in a bid to repurpose the gas for innovative fuel production. Plants can draw electrons from water. These electrons are then used to reduce CO2, allowing the plants to create both short-term energy stores and sugars and starches for longer-term energy storage and structural components. Luckily for humans, oxygen is produced as a by-product of this process.
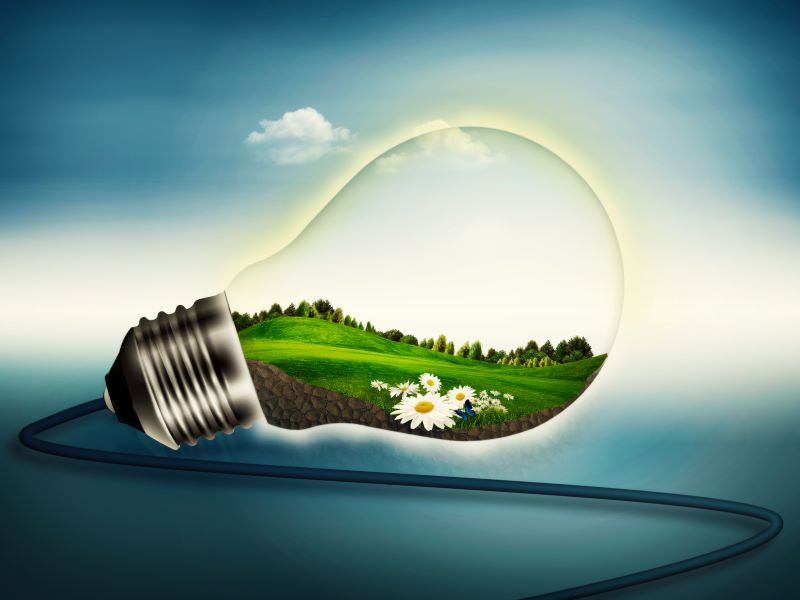
When working in a lab to oxidize water by removing electrons, a molecular catalyst typically fails after about 30 minutes. In contrast, the M.O.F. catalysts have been able to run the test for six hours, and the group feels longer could be possible.
There are many catalysts out there able to make highly-useful carbon monoxide gas through several chemical processes. The removal of one oxygen atom from carbon dioxide, CO2, to create carbon monoxide, CO, is precisely what photosynthesis in plants does. The CO is then converted into other compounds in the cell, usually carbohydrates. However, the problem of using these catalysts is one of selectivity and efficiency– how to get to the required product at suitable efficiency and purity? Dr. Morris expects that rather than a single compound that will replicate photosynthesis, a mix of catalysts will most likely be needed – each taking on a specialized part of the entire process. As it happens, this is similar to how plants do photosynthesis – through a range of proteins. Using this approach of mixed catalysts potentially enables a range of higher-value compounds to be produced from the starting point of artificial photosynthesis.
Sourcing the right solution
There’s a lot of speculation within science communities about the ideal solution for artificial photosynthesis. For our team, water is still proving most popular, combined with altering pH levels to give a range of possible outcomes. Operating at basic pH, water oxidation is far easier. Alter it to operate at acidic levels will make it easier to reduce CO2 but problematically also introduce additional and unwanted reactions. For this reason, the team finds using pure water from the outset preferable rather than later acidifying or basifying the system, giving them the reassurance of first knowing if something works before considering solution conditions.
At ambient pressure, CO2 dissolves in water very poorly, producing a solution with very low levels of CO2. To combat this, the team often works with pressurized systems to increase the amount of dissolved CO2. Typically, room temperature or as low a temperature as possible is preferred, with the maximum elevated temperatures staying around 60 oC. At all temperatures, pressure conditions are closely watched.
Catalytic futures for a greener planet
The use of catalysts to reduce CO2 shows great promise, but each step forward is currently hard-won. The science community hankers for a time when catalysts could combine all of these stages into just one step. Until that’s possible, they are already able to bring them together in cascaded catalysis systems to create superior end products. Under this, a catalyst can react and go on to interface with another, or even a third catalyst, in a joined-up chain of activity. The challenge is that not all catalysts work under the same conditions as others, so unifying them is the issue.
We hope you’ve enjoyed finding out more about the high-pressure catalysis work of Dr Amanda Morris, Associate Chair and Professor of Chemistry, and the Patricia Caldwell faculty fellow and her team at Virginia Tech. For more details, listen to episode 17 of our Modern Chemistry podcast.
Amanda is contactable on social media, and you can find them via
LinkedIn and Twitter.
You can also visit Amanda’s lab page at Virginia Tech, which is a great starting point for more information.
Make sure you read our blog, ‘Better than the real thing? Lab-based photosynthesis hints at new fuel revolution‘ that we think you will also find interesting.